Professors Jain and Sochnikov received NSF research grant entitled “New Quantum Elastocaloric Demagnetization Refrigeration for the Millikelvin Range”. A major focus of their research will be the cooling of quantum chips. For this purpose, their teams will study ‘spin liquids’, which can be harnessed to achieve millikelvin temperatures without magnetic fields. At such low temperatures, quantum phase transitions drive cryocooling. This research uses novel techniques to induce and tune these types of phase transitions. In the future, this research will transform our ability to build energy-efficient, large-scale quantum computers.
CMP
Posts related to condensed matter physics research
Prof. Jain is organizing International Workshop on Oxide Electronics
Associate Professor of Physics Menka Jain and the Institute of Materials Science is co-organizing a workshop-28th International Workshop on Oxide Electronics (IWOE) in Maine next month. The IWOE series has become an important venue to discuss recent advances and emerging trends in this developing field. The aim of the workshop is to provide an interdisciplinary forum for researchers – theorists as well as experimentalists – on understanding the fundamental electronic and structural properties and also on the design, synthesis, processing, characterization, and applications of (epitaxial) functional oxide materials. Results of critical scientific importance as well as studies revealing the technological potential of functional oxide thin films to create devices with enhanced performance will be showcased. The other committee members are: https://iwoe28.events.yale.edu/committees
Dr. Jain designed the logo of the workshop as well.
The full abstract book of the talks and posters can be found at https://iwoe28.events.yale.edu/sites/default/files/files/Abstract%20book_draft.pdf
Two Physicists are in Project Daedalus that Focuses on Materials for Aerospace in New $4.7 Million Contract
UConn’s collaboration with the Department of Defense Air Force Research Laboratory (AFRL) is launching a new project. It is titled Multiscale Modeling and Characterization of Metamaterials, Functional Ceramics and Photonics. This is a $4.7 M contract with $1M for Physics. The project’s goal is to explore and advance the understanding of electronic, photonic, magnetic, and multiferroic materials, with future applications in the aerospace industry. Two experimental condensed matter physicists Dr. Menka Jain and Dr. Ilya Sochnikov will contribute to the understanding of magnetic and multiferroic materials. The project supports 4 graduate Research Assistants in the Physics Department and is a unique life-transformative and career-building opportunity for them.
For more information, see UConn Today article
Stretching Makes Superconductor
October 12, 2020 – Kim Krieger – UConn Communications
When people imagine new materials, they typically think of chemistry. But UConn physicist Ilya Sochnikov has another suggestion: mechanics.
Sochnikov works with superconductors. Superconductors are materials that let electricity flow without losing energy. In a normal conductor — say, a power line — electric current is gradually whittled down by friction and loss. We lose as much as 90% of the electricity we generate this way. But an electric current could flow through a superconducting circuit forever, unchanging. Practical superconductors would make power grids and many devices, including new computers, much more energy efficient.
Chemists and metallurgists have experimented with different combinations of elements for years, trying to get superconductors that work at temperatures close to room temperature (most superconductors only work when they are super cold.) The idea is to come up with the perfect combination of elements that will have exactly the right density of electrons, at the right energies. When that happens, electrons pair up and move through the material in a synchronized way, even at temperatures above 77 degrees Kelvin, which is the temperature of liquid nitrogen. That is considered a high-temperature superconductor, because liquid nitrogen is cheap to produce and can be used as a refrigerant. But finding the right chemistry to make new and better high-temperature superconductors has been elusive.
Sochnikov and his students are thinking about it differently. What if mechanical changes such as squeezing or stretching could make a material a superconductor? Changing the chemistry is ultimately about changing the arrangement of atoms and electrons in a material. Mechanical stresses can do the same thing, in a different way.
Along with Physics Department students Chloe Herrera, Jonah Cerbin, Donny Davino, and Jacob Franklin, Sochnikov designed a machine to stretch a small piece of superconductor to see what would happen. They picked strontium titanate, a well-known material used in high-tech electronics applications as big and almost perfect crystals, which becomes a superconductor around 0.5 degrees Kelvin. That is ridiculously cold, colder even than liquid helium. But strontium titanate behaves in a very weird way when it is that cold. Its atoms polarize; that means they all oscillate in synchrony. You can imagine them bouncing gently up and down, all together. These oscillations have a tendency to link electrons together, helping them move as a pair–this is probably what makes it superconduct.
Sochnikov and the students in the group knew that stretching strontium titanate would change how its atoms oscillated. That, in turn, might change how the electrons moved. The machine that stretches the crystal is made from copper to conduct heat away from the crystal. Most of the rest of the workings are coated in gold to reflect heat from the outside. It uses three cylinders to cool the material; first to the temperature of liquid nitrogen (70K), then liquid helium (4K), then to a boiling mixture of helium-3 and helium-4 (due to weird quantum effects, it is even colder than regular liquid helium–just a few thousandths of Kelvin! Really close to absolute zero!)
The whole setup is suspended in a steel frame that floats on shock absorbers, to prevent any vibrations in the floor from disturbing the experiment.
When Sochnikov, Herrera, Cerbin, Davino, and Franklin did the experiment and looked at the results, they found that stretched strontium titanate becomes superconducting at temperatures 40% higher than normal. That is a huge increase, percentage-wise. They believe it is because stretching the material makes it easier for the atoms to oscillate, gluing the electrons together more firmly. Now, they are working to calculate what made the difference, and plan on testing it in other materials in the near future.
“Usually we control materials chemically. Here, we do it mechanically. This gives us another tool to bring superconductors closer to everyday life, and to discover new functionalities,” Sochnikov says.
This article first appeared online on UConn Today, October 12, 2020.
Breaking Up is Hard To Do (for Electrons in High Temperature Superconductors)
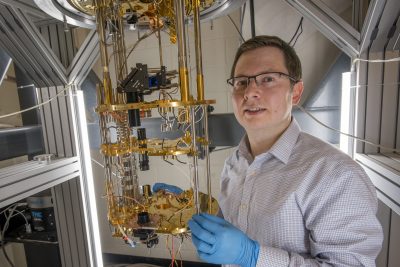
Physicists used to think that superconductivity – electricity flowing without resistance or loss – was an all or nothing phenomenon. But new evidence suggests that, at least in copper oxide superconductors, it’s not so clear cut.
Superconductors have amazing properties, and in principle could be used to build loss-free transmission lines and magnetic trains that levitate above superconducting tracks. But most superconductors only work at temperatures close to absolute zero. This temperature, called the critical temperature, is often only a few degrees Kelvin and requires liquid helium to stay that cold, making such superconductors too expensive for most commercial uses. A few superconductors, however, have a much warmer critical temperature, closer to the temperature of liquid nitrogen (77K), which is much more affordable.
Many of these higher-temperature superconductors are based on a two-dimensional form of copper oxide.
“If we understood why copper oxide is a superconductor at such high temperatures, we might be able to synthesize a better one” that works closer to room temperature (293K), says UConn physicist Ilya Sochnikov.
Sochnikov and his colleagues at Rice University, Brookhaven National Lab and Yale recently figured out part of that puzzle, and they report their results in the latest issue of Nature.
Their discovery was about how electrons behave in copper oxide superconductors. Electrons are the particles that carry electric charge through our everyday electronics. When a bunch of electrons flow in the same direction, we call that an electric current. In a normal electric circuit, say the wiring in your house, electrons bump and jostle each other and the surrounding atoms as they flow. That wastes some energy, which leaves the circuit as heat. Over long distances, that wasted energy can really add up: long-distance transmission lines in the U.S. lose on average 5% of their electricity before reaching a consumer, according to the Energy Information Administration.
But in a superconductor below its critical temperature, electrons behave totally differently. Instead of bumping and jostling, they pair up and move in sync with the other electrons in a kind of wave. If electrons in a normal current are a rushing, uncoordinated mob, electrons in a superconductor are like dancing couples, gliding across the floor like people in a ballroom. It’s this friction-free dance – coherent motion – of paired electrons that makes a superconductor what it is.
The electrons are so happy in pairs in a superconductor that it takes a certain amount of energy to pull them apart. Physicists can measure this energy with an experiment that measures how big a voltage is needed to tear an electron away from its partner. They call it the ‘gap energy’. The gap energy disappears when the temperature rises above the critical temperature and the superconductor changes into an ordinary material. Physicists assumed this is because the electron pairs have broken up. And in classic, low-temperature superconductors, it’s pretty clear that that’s what’s happening.
But Sochnikov and his colleagues wanted to know whether this was really true for copper oxides. Copper oxides behave a little differently than classic superconductors. Even when the temperature rises well above the critical level, the energy gap persists for a while, diminishing gradually. It could be a clue as to what makes them different.
The researchers set up a version of the gap energy experiment to test this. They made a precise sandwich of two slices of copper oxide superconductor separated by a thin filling of electrical insulator. Each slice was just a few nanometers thick. The researchers then applied a voltage between them. Electrons began to tunnel from one slice of copper oxide to the other, creating a current.
By measuring the noise in that current, the researchers found that a significant number of the electrons seemed to be tunneling in pairs instead of singly, even above the critical temperature. Only about half the electrons tunneled in pairs, and this number dropped as the temperature rose, but it tapered off only gradually.
“Somehow they survive,” Sochnikov says, “they don’t break fully.” He and his colleagues are still not sure whether the paired states are the origin of the high-temperature superconductivity, or whether it’s a competing state that the superconductor has to win out over as the temperature falls. But either way, their discovery puts a constraint on how high temperature superconductors happen.
“Our results have profound implications for basic condensed matter physics theory,” says co-author Ivan Bozovic, group leader of the Oxide Molecular Beam Epitaxy Group in the Condensed Matter Physics and Materials Science Division at the U.S. Department of Energy’s Brookhaven National Laboratory and professor of applied physics at Yale University. Sochnikov agrees.
“There’s a thousand theories about copper oxide superconductors. This work allows us to narrow it down to a much smaller pool. Essentially, our results say that any theory has to pass a qualifying exam of explaining the existence of the observed electron pairs,” Sochnikov says. He and his collaborators at UConn, Rice University, and Brookhaven National Laboratory plan to tackle the remaining open questions by designing even more precise materials and experiments.
The research work at UConn was funded by the State of Connecticut through laboratory startup funds.
This article first appeared on UConn Today, August 21, 2019.
Physics students boost interdisciplinary research in materials
By Amanda Olavarria
The Electronic and Advanced Materials Conference (EAM) is geared towards engineers, technologists, researchers and students with an interest in science, engineering and the applications of electroceramic materials. Several MSE students and faculty attended this year’s EAM Conference held in Orlando, FL.
MSE Associate Professor and Director for Undergraduate Studies, Serge Nakhmanson, co-organized a symposium at this event entitled “Mesoscale Phenomena in Ceramic Materials.” Four UConn students including Tulsi Patel, Krishna Chaitanya Pitike, Lukasz Kuna and Hope Whitlock showcased their research.
In addition to the oral presentations, two UConn students claimed 2nd and 3rd place in the American Ceramics Society (ACerS) Electronics Division “Best Student Poster Presentation” awards. Lukasz Kuna received 3rd place for his poster entitled, “Mesoscale Simulations of the Influence of Elastic Strains on the Optical Properties of Semiconducting Core-Shell Nanowires.” Krishna Chaitayna Pitike won 2nd place for his poster, “Shape and Size Dependent Phase Transformations and Field-induced Behavior in Ferroelectric Nanoparticles.”
In response to the latter award Serge Nakhmanson said, “This remarkable work involves contributions from five UConn students (including Physics undergraduate Hope Whitelock) and an exchange student from China visiting my group. It started as a team project in the “Phase Transformations in Solids” graduate class (MSE 5305). Since the original results appeared to be significant, we decided to continue this project beyond the end of the semester to generate a publication for a peer-review scientific journal. This is now being finalized for submission. It is relatively rare to see classroom projects successfully transition into publication quality research, but this one is being well received by the community.” Department Head Bryan Huey adds, “Devising a class project that can be guided through to a publication is a testament to Professor Nakhmanson’s commitment to teaching and the hard work he inspires with these bright students.”
EAM, jointly arranged by the Electronics Division and Basic Science Division of the ACerS, focuses on the properties and processing of ceramic and electroceramic materials and their applications in electronic, electro/mechanical, dielectric, magnetic, and optical components and devices and systems.
Categories: awards, conferences, news, research, students
Published: February 16, 2018
Undergraduate achievements receive wide attention
Thermal Funkiness: Explaining the Unexpected
Whoever said rules were made to be broken wasn’t a physicist. When something doesn’t act the way you think it should, either the rules are wrong, or there’s new physics to be discovered. Which is exactly what UConn’s Connor Occhialini ’18 (CLAS), an honors student majoring in physics and math, found when he began researching scandium fluoride.
Scandium fluoride is a transparent crystal with a cubic shape, a byproduct of mining. It’s not used commercially and it wouldn’t be particularly interesting to anyone except for one odd thing: it shrinks as it warms.
Most materials swell as they heat up. Really simple materials like hydrogen gas swell because the heat makes their atoms zoom around faster, bumping into each other more, so the same number of hydrogen atoms need more space. More complicated materials also swell, which is why your wooden front door tends to stick in the summertime. But solids like wood can’t swell as much as a gas because their atoms are tightly linked together into long, interlocked molecules, so they just jiggle around, swelling the door a little bit.
Scandium fluoride must be doing something else, reasoned Occhialini. His advisor for his honors physics project, Jason Hancock, had been working with scandium fluoride, and asked Occhialini to study a model of the crystal’s dynamics. Scandium fluoride has a pretty simple structure: it’s a solid crystal, with each scandium atom surrounded by six fluorines to make stacks of octahedra (eight-sided diamonds). The researchers hoped the simple structure might be easy to understand. Understanding scandium fluoride’s strange ‘negative thermal expansion’, as physicists call the heat-related shrinkage, might yield more general insight into other, more complex materials that do the same thing.
Occhialini’s first step was to simplify the problem. So instead of a three-dimensional crystal, he decided to think about it as a two-dimensional sheet that looks like this:
Each black diamond represents a molecule of scandium fluoride. The scandium atoms (blue dots) are at the center of each diamond, and a fluorine atom is at each corner.
Most of the time, bonds between atoms are flexible. So in a normal crystalline solid – calcium fluoride, for example – the fluorines and calcium atoms would all be able to wiggle around independently when the material warmed up. As they wiggled, they’d take up a little more space, and the solid would swell. Normal solid behavior.
But Occhialini wondered if maybe that wasn’t what was happening in scandium fluoride. Maybe in this model, he should assume the bonds connecting each fluorine to its scandium were stiff? So stiff the fluorine-scandium bonds don’t move at all, so the diamonds are like solid blocks. The only places the structure would be able to flex when it warmed up would be at the fluorine atoms, which would act like tiny little joints. As the crystal heated up, the little scandium fluoride blocks would tilt around the fluorines at the corners. That’s what you see happening in the picture. You’ll notice that when the diamonds tilt, the whole structure gets smaller. It actually tightens up. The blue outline shows the structure at its coldest, perfectly ordered state, with no molecular motion. When the diamonds tilt, they take up a smaller total volume than the blue outline delineates. This is negative thermal expansion.
Occhialini figured out that you can describe this shrinkage mathematically, using just the angle of the molecules’ tilt. He called the angle Θ (theta). When the scandium fluoride blocks tilt by an angle Θ, the distance between the center of each block shortens by a factor of cosine Θ, and the crystal’s total volume shrinks.
To calculate that shrinkage (or, in a normal material, expansion) in detail, Occhialini added a third term to the classic equation that describes the energy of a vibrating crystal. The first two terms in the standard equation describe the potential energy a crystal has from the bending at each molecular junction, plus the kinetic energy of rotation of each molecule. Occhialini’s equation also describes the translational kinetic energy of the molecules–not just from rotating around, but also moving toward and away from their original positions as they rotate. The further they are from the center of mass of the crystal, the more they move. Look back at Figure 1 and notice the dot in the middle; that’s the center of mass. The diamonds in the middle barely move in relation to it, while the diamonds at the edges move a lot. Now imagine how much of a difference there would be if the crystal had millions of molecules instead of just 25. And now you understand how important that third term could be to the energy of the crystal.
Now, molecules being molecules, they don’t just shrink and stay there. They’re moving constantly, and the warmer they get, the more they move. Part of Occhialini’s insight is that, on average, the molecular structure gets bendier the warmer it gets. So the molecules tilt more and spend more time at bigger values of Θ, closer to 45 degrees. After Occhialini thought it over for a while together with Hancock and physics Ph.D. students Sahan Handunkanda and Erin Curry, they realized there was a geometric shape that had the same mathematical description. It’s Archimedes’ spiral pendulum, and it looks like this:
Each turning of the spiral is exactly the same distance from the last. That spacing – the distance between turns – is controlled by Θ. Imagine a line that stretches from the center of the sphere to a point on the spiral. The angle between that line and the pole of the sphere is Θ. You see the little ball traveling along the spiral? That’s the end of the imaginary line. As Θ gets bigger, the ball moves towards the equator. Imagine that the ball represents the instantaneous state of the scandium fluoride crystal – the physicists calculated the statistical average of what every molecule in the crystal is doing. You’ll notice the ball spends more time near the equator of the spiral sphere, that is, it tends to hang out where Θ is large. If the temperature of the crystal drops and the molecules wiggle less, Θ gets smaller, the more time the ball spends near the pole of the sphere and the less the crystal shrinks.
So not only can a really weird phenomenon of a crystal that shrinks as it warms be explained by just assuming the molecules are rigid, but it can be illustrated with a classical geometric shape!
Occhialini was just a freshman when Hancock introduced him to the scandium fluoride puzzle. He had to learn the math as he went, but after about two semesters of working on it he’d figured out the equation that described what was going on. Now in his senior year, he says his research experiences in Hancock’s lab have been integral to his experience as an undergraduate.
The equation works beautifully and explains certain aspects of Hancock’s experimental x-ray measurements as well.
“I learned a lot more doing research than any course could have given me,” Occhialini says.
And now you, dear reader, have learned a little bit, too.
Occhialini’s research has been funded through his advisor’s NSF grant no. DMR-1506825 and a SURF fellowship from the Office of Undergraduate Research. He was also named a Treibick Scholar.
Physics student John Mangeri wins prestigious fellowship
John Mangeri’s Award Lands Him in Argonne National Laboratory
John Mangeri (left) with his SCGSR-award host Dr. Olle Heinonen (right) in front of the Chemistry building (bldg. 200) at Argonne National Laboratory.
(Photo credit to Dr. Andrea Jokisaari)
By Katherine Eastman
John Mangeri, a Ph.D. candidate in Dr. Serge Nakhmanson’s “Complex Materials by Computational Design” group, was selected to receive the U.S. Department of Energy’s Office of Science Graduate Research (DoE SCGSR) award for his project, Computational Design of Functional Materials for Electrothermal Energy Interconversion on Mesoscale.
This award allowed John to conduct research on his project at the Argonne National Laboratory in Lemont, IL, for from June to September in 2016 under the guidance of the DoE collaborator, Dr. Olle G. Heinonen.
The U.S. Department of Energy states that the “SCGSR program provides supplemental awards to outstanding U.S. graduate students to pursue part of their graduate thesis research at a DoE laboratory in areas that address specific challenges central to the Office of Science mission.”
Argonne National Laboratory is one of the U.S. Department of Energy’s premier national laboratories for scientific and engineering research. Its state-of-the-art, high-performance computing facilities that were available to John during his visit enabled him to achieve rapid progress in advancing his Ph.D. project.
“I am extremely pleased with John’s research accomplishments on the way to his Ph.D. degree. John is currently the main code developer for the mesoscale-level multiphysics simulation package, ‘Ferret,’ that is being utilized by the group together with our Argonne collaborators to design new materials that can convert thermal energy into electrical and vice versa,” Dr. Nakhmanson commented.
John’s research on a new material concept for this energy conversion by utilizing an electrocaloric effect that changes the temperature of a dielectric when subjected to an external electric field was recently published in a new journal, NPJ Computational Materials, that is partnered with the prestigious scientific journal Nature. The article, entitled “Amplitudon and phason modes of electrocaloric energy interconversion,” was co-authored by John, Krishna Pitike (also a graduate student in Dr. Nakhmanson’s group), Dr. Pamir Alpay, and Dr. Nakhmanson.
In that project, the co-authors conducted a theoretical investigation of a model system made up of thin perovskite-oxide crystal layers, whose polarization directions can be easily reoriented by an applied electric field.
This unusual system, the team demonstrated, must exhibit two different kinds of electrocaloric responses, conventional and anomalous one, that can either heat the material up or cool it down with a capability to switch between these two modes on demand. Possible applications for this effect are new, integrated cooling sources for computer chips and other electronic circuits, as well as more efficient and silent HVAC devices.
“The effect we saw was quite unexpected. We were able to show that there are two kinds of energy conversion modes in that material — stemming, respectively, from either amplitudon or phason excitations of the local polar dipoles,” John said.
Even though this material does not yet exist, he further explained, quantum mechanics suggests that it could be put together by one of atomic layer-by-layer deposition techniques that are utilized for growing thin oxide films on substrates.
“It’s a good opportunity for me,” John said in reflection of his SCGSR-sponsored research experience at Argonne. “There’s always more work to do — you always have to be looking at the next step in developing your career and being exposed to a different setting for doing science really helps with evaluating your priorities.”
Prof. Sochnikov is a recipient of Montana Instruments Cold Science Exploration Awards
Dr. Sochnikov is a recipient of Montana Instruments Cold Science Exploration Awards Lab Startup Grant.
Dr. Ilya Sochnikov has just started new scanning SQUID microscopy lab at the University of Connecticut.
Ilya Sochnikov’s research focuses on nanoscale quantum phenomena in new materials. An emergence of a new phenomenon or a phase transition occurs when interactions in the materials are tuned via chemical, mechanical, or electromagnetic knobs. The material systems of an immediate interest include topological insulators, superconductors, and frustrated magnets. His main research tool will be a state of the art microscope for imaging of tiny magnetic fields at ultra-low temperatures and short timescales. One of the research motivations is to impact our understanding of materials properties that could provide new options for energy efficient technologies.
“Caution: Shrinks When Warm”
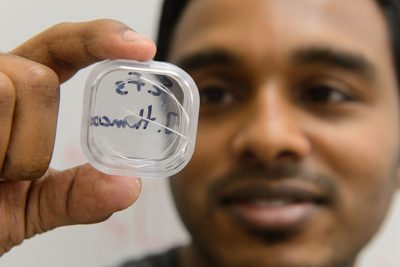
Kim Krieger – UConn Communications
–Jason Hancock, Assistant Professor in Physics, with graduate students, Erin Curry and Sahan Handunkanda, have been investigating a substance that shrinks when it warms.
Most materials swell when they warm, and shrink when they cool. But UConn physicist Jason Hancock has been investigating a substance that responds in reverse: it shrinks when it warms.
Although thermal expansion, and the cracking and warping that often result, are an everyday occurrence – in buildings, bridges, electronics, and almost anything else exposed to wide temperature swings – physicists have trouble explaining why solids behave that way.
Research by Hancock and his colleagues into scandium trifluoride, a material that has negative thermal expansion, recently published in Physical Review B, may lead to a better understanding of why materials change volume with temperature at all, with potential applications such as more durable electronics. For the complete article in UConn Today that explains their findings, see “Caution: Shrinks When Warm” .